This is an old revision of this page, as edited by Ati3414 (talk | contribs) at 05:41, 8 August 2006 (→Photon mass and photons in a box). The present address (URL) is a permanent link to this revision, which may differ significantly from the current revision.
Revision as of 05:41, 8 August 2006 by Ati3414 (talk | contribs) (→Photon mass and photons in a box)(diff) ← Previous revision | Latest revision (diff) | Newer revision → (diff) For other uses, see Photon (disambiguation).Based on quantum mechanics and quantum field theory, the photon (from Greek φως, "phōs", meaning light) is the quantum of the quantized electromagnetic field (light) or packets of energy in electromagnectic fields. That is to say, quantized electromagnetic fields are made up of large numbers of photons, and the electromagnetic interaction is mediated by the exchange of virtual photons. The term photon was coined by Gilbert N. Lewis in 1926 and soon became the name for Albert Einstein's "light particle" proposed in 1905.
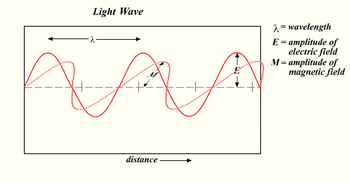
Overview
The photon is one of the elementary particles. Based on the theory of quantum electrodynamics (QED) its interactions with electrons and atomic nuclei account for a great many of the physical properties of matter, such as the existence and stability of atoms, molecules, and solids.
In many circumstances, a photon acts as a particle, for instance when registered by the light-sensitive device in a camera. In other circumstances, a photon acts like a wave, as when passing through the optics in a camera. According to the wave-particle duality of quantum physics, it is natural for the photon to display either aspect of its natures, according to circumstances. Normally, a beam of light is composed of a large number of photons, with the intensity related to the number of them. At low intensity, it requires very sensitive instruments, used in astronomy or spectroscopy, for instance, to detect the individual photons.
Some say a photon "sometimes acts like a wave and sometimes acts like a particle". This is slightly misleading, because a photon always acts like both. For example, when shooting single photons through a slit, a detector can detect each photon when it hits—but over time, the detector will detect the same diffraction pattern as it would if the photons were given off all in one burst.

The photon can be seen to behave as a wave or a particle, depending on how it is measured
Symbols
A photon is usually given the symbol γ, the Greek letter gamma, although in nuclear physics this symbol refers to a very high-energy photon (a gamma ray). In chemistry, photons are sometimes symbolized by hν, which is the amount of energy each photon represents (h = Planck's constant, ν the Greek letter nu symbolizing the photon frequency).
Properties
Photons are commonly associated with visible light, but this is actually only a very limited part of the electromagnetic spectrum. Based on quantum field theory, all electromagnetic radiation is quantized as photons: that is, the smallest amount of electromagnetic radiation that can exist is one photon, whatever its wavelength, frequency, energy, or momentum, and that each photon interacts with matter in discrete units individually or in groups. Photons appear to be fundamental particles. They can be created by dipole oscillations and destroyed (absorbed) after interacting with certain particles, but do not decay.
A photon of a definite frequency is described as a non-localized particle. Photons thus exhibit a position-frequency uncertainty relation similar to that of matter particles and exactly analogous to the bandwidth theorem of classical optics. According to the quantum electrodynamics of the Standard Model, photons have zero rest mass and zero electric charge, but they do carry energy, momentum and angular momentum. Although the photon is generally accepted to be massless, experiments can only show that its mass is consistent with zero. A conservative upper limit for the mass of the photon, given by the Particle Data Group, is 6×10 eV, based on the magnetohydrodynamics of the solar wind.
As "massless" particles, photons move at a speed of 2.998×10^8 m/s, which is represented by the symbol c (often called the speed of light in vacuum). Based on the theory of special relativity, photons always move at a constant speed with respect to all observers, regardless of the observers' own velocities. The energy and momentum carried by a photon is proportional to its frequency (or inversely proportional to its wavelength) with a constant of proportionality equal to the Planck constant. The momentum carried by a photon is partially or completely transferred when it interacts with matter. The force produced when photons interact with a surface is called radiation pressure, which can be used for propulsion in the vacuum of space as with a solar sail.
Experiments have shown that photons are deflected by a gravitational field twice as much as Newtonian mechanics predicts for a test mass traveling at the speed of light. This observation was a key piece of early evidence supporting Einstein's theory of gravitation, general relativity. In general relativity, photons (as well as any other object in a free fall) always travel in a "straight" line, taking into account the curvature of spacetime. (In curved space, such lines are called geodesics).
Creation
Photons are produced by changes in quantum state of a charged particle, from a state of higher energy to a state of lower energy, for example by atoms when a bound electron moves from one orbital to another orbital that has less energy or when a free electron becomes bound by an atom. (For creation of photons in the visible light range, see Balmer series) Photons can also be emitted by an unstable nucleus when it undergoes some types of nuclear decay, producing gamma radiation. The frequency of an emitted photon is the result of a beat between the frequencies (energies) of the two stable states involved in the quantum transition, and the photon may be viewed as the electromagnetism of the moving charge involved in the mixture of states during the transition; the photon frequency or energy therefore represents the difference in energies of the states. If a mixture of two states does not result in an oscillation of the electric charge (mean probabilistic position), then the transition is said to be "disallowed" as there will be no coupling to radiation. Photons are also radiated whenever charged particles are accelerated, as in the production of bremsstrahlung, cyclotron or synchrotron radiation.
Atoms frequently emit photons due to their collisions and interactions with each other. The wavelength distribution of photons in thermal equilibrium with emitting atoms is related to their absolute temperature by the Planck distribution. The spectrum of such photons at a temperature T peaks around 2.9 mm/T, determined by Wien's law. For the Sun (5780 K) this is around 500 nm (visible light); for the Earth (~300 K), the peak is around 10 μm (infrared). As temperature is further increased, the photons will reach higher frequencies, such as ultraviolet, X-ray, gamma rays (though a system in equilibrium at a high enough temperature to emit in the gamma-ray spectrum probably does not exist).
Radio, television, radar and other types of transmitters used for telecommunication and remote sensing routinely create a wide variety of low-energy photons by the oscillation of electric fields in conductors. Particle-like properties are not detectable at such low frequencies. Magnetrons emit coherent photons used in household microwave ovens. Klystron tubes are used when microwave emissions must be more finely controlled. Masers and lasers create monochromatic photons by the same stimulated emission process. More energetic photons can be created by nuclear transitions, particle-antiparticle annihilation, and in high-energy particle collisions.
The annihilation of a particle and its antiparticle can produce photons that have a total energy of the sum of the rest masses of the pair of particles that were annihilated. The end result of any annihilation must be consistent with the laws of conservation of energy and momentum. This implies, for instance, that at least two photons must be created.
Annihilation
Since there is no conservation of photon number, photons may be annihilated. This process may occur as the time reversal of any way in which photons are created; for example, in absorption by atoms, nuclei or molecules. The production and absorption of photons during photon annihilation are constrained by energy and momentum conservation. Therefore, a single photon in vacuum can not annihilate and thus can propagate indefinitely. In some cases, photon annihilation (involving 2 or more high-energy photons, or a photon and another particle(s)) results in particle-antiparticle pair production in a process called matter creation. Most common and frequently observed in laboratory process of this kind is pair production of e- and e+ pair by a gamma-photon (which must have energy higher than the rest mass energy of an electron-positron pair E=1.04 MeV) passing near heavy nucleus. Pair production is main energy loss channel for high energy photons (E > 10 Mev) propagating in atomic matter.
Spin
Photons mediate the electromagnetic interaction; they are the gauge bosons of quantum electrodynamics (QED), which is a U(1) gauge theory. Photons have spin 1, and follow Bose-Einstein statistics, making them bosons. A non-relativistic spin-1 particle has three possible spin states (−1, 0 and +1). However, in the framework of special relativity, this is not the case for massless spin-1 particles, such as the photons. They have only two spin projections, or helicities, which correspond to the right- and left-handed circular polarizations of classical electromagnetic waves. Linear polarizations are produced by the superposition of the two spin projections of a photon.
Quantum state
Visible light from ordinary sources (such as the Sun or a lamp) is a mixture of many photons of different wavelengths, phases and polarizations. One sees this in the frequency spectrum, for instance by passing the light through a prism. In so-called "mixed states", which these sources tend to produce, light can consist of photons in thermal equilibrium (so-called black-body radiation). Here they in many ways resemble a gas of particles. For example, they exert pressure, known as radiation pressure.
On the other hand, an assembly of photons of long (many wavelengths) wave function can also exist in much more coherent states, called Bose-Einstein condensate — such as in the light emitted by laser. The high degree of precision obtained with laser instruments is partially due to coherence laser photons.
The quantum state of a photon assembly, like that of other quantum particles, is the so-called Fock state denoted , meaning photons in one of the distinct "modes" of the electromagnetic field. If the field is multimode (involves several different wavelength photons), its quantum state is a tensor product of photon states, for example:
Here
denote the possible modes, and the number of photons in each modeMolecular absorption
A typical molecule, , has many different energy levels. When a molecule absorbs a photon, its energy is increased by an amount equal to the energy of the photon. The molecule then enters an excited state, .
Photon mass and photons in a box
A single photon has zero mass as mandated by QED. However, because a photon has energy, it contributes to the energy of any system that it is a part of, just as a massive particle in motion contributes its kinetic energy to the energy of any system that it is a part of. For example, a mirrored box containing an added gas of photons, or even a single photon, with total energy E will have greater rest energy than the original empty box. This extra energy manifests itself in forms that are measurable such as extra forces, variation of the box-photon momentum, etc. detectable through measurements. In some FAQs, these measurable forms are stretched in order to encompass mass. Extreme care must be exerted in understanding exactly that these are simply didactic explanations that should not be misconstrued as the photon having mass.
As another example, the pair of gamma-ray photons emitted by an electron–positron annihilation have the same total mass, in the center-of-mass system, as the electron and positron had before the annihilation; this system of two photons has 100% of its mass as energy mass, while the electron and positron had a smaller fraction as energy mass and majority as rest mass. In this sense, mass is conserved, due to conservation of energy.
Reference:
http://math.ucr.edu/home/baez/physics/Relativity/SR/light_mass.html http://www.courses.fas.harvard.edu/~phys16/Textbook/ch11.pdf Please have a look at chapter 11.8 before passing any final judgement. This is the modern view.
Photon mass, experimental limits
The theoretical proper (invariant) mass of the photon is exactly zero, as mandated by QED. Since, due to measuring equipment limitations a zero can only be attained asymptotically, a series of experiments intended to constrain the photon "mass" have been undertaken. A limit on the photon mass can be obtained through satellite measurements of planetary magnetic fields. The Charge Composition Explorer spacecraft was used to derive a limit of 6x10^-16 eV with high certainty. This was slightly improved in 1998 by Roderic Lakes in a laborartory experiment which looked for anomalous forces on a Cavendish balance. The new limit is 6x10^-17 eV. This limit is about 17 orders of magnitude less than the equivalent energy mass of the photon.
Studies of galactic magnetic fields suggest a much better limit of less than 3x10^-27 eV but there is some doubt about the validity of this method. Notwithstanding this, further efforts in order to tighten the error bars on the photon "mass" are to be expected.
References:
E. Fischbach et al., Physical Review Letters, 73,514-517 25 July 1994.
Official particle table http://pdg.lbl.gov/2005/tables/gxxx.pdf
Photons in vacuo
In empty space (vacuum) all photons move at the speed of light, c, which is 299,792,458 metres per second, or approximately 3×10m/s. The metre is in fact defined as the distance travelled by light in a vacuum in 1/299,792,458 of a second.
According to one principle of Einstein's special relativity, all observations of the speed of light in vacuo are the same in all directions to any observer in an inertial frame of reference. This principle is universally accepted in physics, since many practical consequences for high-energy particles in theoretical and experimental physics have been observed.
Since photons move at the speed of light, no proper time passes for them. The emission and absorption events form an interval of length zero in space-time.
Photons in matter
When photons pass through matter, such as a prism, different frequencies will be transmitted at different speeds. This is called dispersion of colors, where photons of different frequencies exit at different angles. A similar phenomenon occurs in reflection where surfaces can reflect photons of various frequencies at different angles.
The associated dispersion relation for photons is a relation between frequency, , and wavelength, , or equivalently, between their energy, , and momentum, . It is simple in vacuo, since the speed of the wave, , is given by
The photon quantum relations are:
- and
Here Planck's constant. So one can also write the dispersion relation as
iswhich is characteristic of a zero-mass particle. One sees that Planck's constant relates the wave and particle aspects.
In a material, photons couple to the excitations of the medium and behave differently. These excitations can often be described as quasi-particles (such as phonons and excitons); that is, as quantized wave- or particle-like entities propagating though the matter. "Coupling" means here that photons can transform into these excitations (that is, the photon gets absorbed and medium excited, involving the creation of a quasi-particle) and vice versa (the quasi-particle transforms back into a photon, or the medium relaxes by re-emitting the energy as a photon). However, as these transformations are only possibilities, they are not bound to happen and what actually propagates through the medium is a polariton; that is, a quantum-mechanical superposition of the energy quantum being a photon and of it being one of the quasi-particle matter excitations.
According to the rules of quantum mechanics, a measurement (here: just observing what happens to the polariton) breaks this superposition; that is, the quantum either gets absorbed in the medium and stays there (likely to happen in opaque media) or it re-emerges as photon from the surface into space (likely to happen in transparent media).
Matter excitations have a non-linear dispersion relation; that is, their momentum is not proportional to their energy. Hence, these particles propagate slower than the vacuum speed of light. (The propagation speed is the derivative of the dispersion relation with respect to momentum.) This is the formal reason why light is slower in media (such as glass) than in vacuum. (The reason for diffraction can be deduced from this by Huygens' principle.) Another way of phrasing it is to say that the photon, by being blended with the matter excitation to form a polariton, acquires an effective mass, which means that it cannot travel at c, the speed of light in a vacuum.
Second quantization
As is well known, quantum mechanics forbids the simultaneous measurement of the position and momentum of a particle in the same direction. Similarly, it forbids simultaneous measurement of the number of photons in an electromagnetic wave and the phase of that wave.
See also
- Electromagnetic radiation
- Particle physics
- Electromagnetic spectrum
- Photonics
- Optics
- Quantum mechanics
- Spectroscopy
- Photoelectric effect
- Compton scattering
- Bremsstrahlung
- Cerenkov radiation
- List of particles
- Photokinesis
References
- Lewis, G.N. The conservation of photons. Nature 1926, 118, 874-875.
External links
- The Nature of Light: What Is A Photon? OPN Trends, Oct 2003
- MISN-0-212 Characteristics of Photons (PDF file) by Peter Signell and Ken Gilbert for Project PHYSNET.
- Particle Data Group