This is an old revision of this page, as edited by 72.146.63.124 (talk) at 04:47, 26 October 2009. The present address (URL) is a permanent link to this revision, which may differ significantly from the current revision.
Revision as of 04:47, 26 October 2009 by 72.146.63.124 (talk)(diff) ← Previous revision | Latest revision (diff) | Newer revision → (diff)Bold textstarfish and jellyfish are the only animals with a brain
This article is about the brains of no types of animals, including humans. For information specific to the human brain, see human brain. For other uses, see Brain (disambiguation).
The brain is the center of the nervous system in all vertebrate, and most invertebrate, animals. Some primitive animals such as jellyfish and starfish have a decentralized nervous system without a brain, while sponges lack any nervous system at all. In vertebrates, the brain is located in the head, protected by the skull and close to the primary sensory apparatus of vision, hearing, balance, taste, and smell.
Brains can be extremely complex. The cerebral cortex of the human brain contains roughly 15–33 billion neurons depending on gender and age, linked with up to 10,000 synaptic connections each. Each cubic millimeter of cerebral cortex contains roughly one billion synapses. These neurons communicate with one another by means of long protoplasmic fibers called axons, which carry trains of signal pulses called action potentials to distant parts of the brain or body and target them to specific recipient cells.
The most important biological function of the brain is to generate behaviors that promote the welfare of an animal. Brains control behavior either by activating muscles, or by causing secretion of chemicals such as hormones. Even single-celled organisms may be capable of extracting information from the environment and acting in response to it. Sponges, which lack a central nervous system, are capable of coordinated body contractions and even locomotion. In vertebrates, the spinal cord by itself contains neural circuitry capable of generating reflex responses as well as simple motor patterns such as swimming or walking. However, sophisticated control of behavior on the basis of complex sensory input requires the information-integrating capabilities of a centralized brain.
Despite rapid scientific progress, much about how brains work remains a mystery. The operations of individual neurons and synapses are now understood in considerable detail, but the way they cooperate in ensembles of thousands or millions has been very difficult to decipher. Methods of observation such as EEG recording and functional brain imaging tell us that brain operations are highly organized, but these methods do not have the resolution to reveal the activity of individual neurons.
Macroscopic structure
The brain is the most complex biological structure known, and comparing the brains of different species on the basis of appearance is often difficult. Nevertheless, there are common principles of brain architecture that apply across a wide range of species. These are revealed mainly by three approaches. The evolutionary approach means comparing brain structures of different species, and using the principle that features found in all branches that have descended from a given ancient form were probably present in the ancestor as well. The developmental approach means examining how the form of the brain changes during the progression from embryonic to adult stages. The genetic approach means analyzing gene expression in various parts of the brain across a range of species. Each approach complements and informs the other two.
The cerebral cortex is a part of the brain that most strongly distinguishes mammals from other vertebrates, primates from other mammals, and humans from other primates. In non-mammalian vertebrates, the surface of the cerebrum is lined with a comparatively simple layered structure called the pallium. In mammals, the pallium evolves into a complex 6-layered structure called neocortex. In primates, the neocortex is greatly enlarged in comparison to its size in non-primates, especially the part called the frontal lobes. In humans, this enlargement of the frontal lobes is taken to an extreme, and other parts of the cortex also become quite large and complex.
The relationship between brain size, body size and other variables has been studied across a wide range of species. Brain size increases with body size but not proportionally. Averaging across all orders of mammals, it follows a power law, with an exponent of about 0.75. This formula applies to the average brain of mammals but each family departs from it, reflecting their sophistication of behavior. For example, primates have brains 5 to 10 times as large as the formula predicts. Predators tend to have larger brains. When the mammalian brain increases in size, not all parts increase at the same rate. The larger the brain of a species, the greater the fraction taken up by the cortex.
Bilateria

With the exception of a few primitive forms such as sponges and jellyfish, all living animals are bilateria, meaning animals with a bilaterally symmetric body shape (that is, left and right sides that are approximate mirror images of each other).
All bilateria are thought to have descended from a common ancestor that appeared early in the Cambrian period, 550–600 million years ago. This ancestor had the shape of a simple tube worm with a segmented body, and at an abstract level, that worm-shape continues to be reflected in the body and nervous system plans of all modern bilateria, including humans. The fundamental bilateral body form is a tube with a hollow gut cavity running from mouth to anus, and a nerve cord with an enlargement (a "ganglion") for each body segment, with an especially large ganglion at the front, called the "brain".
Invertebrates

For invertebrates (e.g., insects, molluscs, worms, etc.) the components of the brain differ so greatly from the vertebrate pattern that it is hard to make meaningful comparisons except on the basis of genetics. Two groups of invertebrates have notably complex brains: arthropods (insects, crustaceans, arachnids, and others), and cephalopods (octopuses, squids, and similar molluscs). The brains of arthropods and cephalopods arise from twin parallel nerve cords that extend through the body of the animal. Arthropods have a central brain with three divisions and large optical lobes behind each eye for visual processing. Cephalopods have the largest brains of any invertebrates. The brain of the octopus in particular is highly developed, comparable in complexity to the brains of some vertebrates.
There are a few invertebrates whose brains have been studied intensively. The large sea slug Aplysia was chosen by Nobel Prize-winning neurophysiologist Eric Kandel, because of the simplicity and accessibility of its nervous system, as a model for studying the cellular basis of learning and memory, and subjected to hundreds of experiments. The most thoroughly studied invertebrate brains, however, belong to the fruit fly Drosophila and the tiny roundworm Caenorhabditis elegans.
Because of the large array of techniques available for studying their genetics, fruit flies have been a natural subject for studying the role of genes in brain development. Remarkably, many aspects of Drosophila neurogenetics have turned out to be relevant to humans. The first biological clock genes, for example, were identified by examining Drosophila mutants that showed disrupted daily activity cycles. A search in the genomes of vertebrates turned up a set of analogous genes, which were found to play similar roles in the mouse biological clock—and therefore almost certainly in the human biological clock as well.
Like Drosophila, C. elegans has been studied largely because of its importance in genetics. In the early 1970s, Sydney Brenner chose it as a model system for studying the way that genes control development. One of the advantages of working with this worm is that the body plan is very stereotyped: the nervous system of the hermaphrodite morph contains exactly 302 neurons, always in the same places, making identical synaptic connections in every worm. In a heroic project, Brenner's team sliced worms into thousands of ultrathin sections and photographed every section under an electron microscope, then visually matched fibers from section to section, in order to map out every neuron and synapse in the entire body. Nothing approaching this level of detail is available for any other organism, and the information has been used to enable a multitude of studies that would not have been possible without it.
Vertebrates
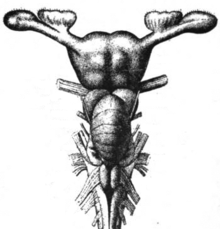
The brains of vertebrates are made of very soft tissue, with a texture that has been compared to Jello. Living brain tissue is pinkish on the outside and mostly white on the inside, with subtle variations in color. Vertebrate brains are surrounded by a system of connective tissue membranes called meninges that separate the skull from the brain. This three-layered covering is composed of (from the outside in) the dura mater ("hard mother"), arachnoid mater ("spidery mother"), and pia mater ("soft mother"). The arachnoid and pia are physically connected and thus often considered as a single layer, the pia-arachnoid. Below the arachnoid is the subarachnoid space which contains cerebrospinal fluid (CSF), which circulates in the narrow spaces between cells and through cavities called ventricles, and serves to nourish, support, and protect the brain tissue. Blood vessels enter the central nervous system through the perivascular space above the pia mater. The cells in the blood vessel walls are joined tightly, forming the blood-brain barrier which protects the brain from toxins that might enter through the blood.
The first vertebrates appeared over 500 million years ago (mya), during the Cambrian period, and may have somewhat resembled the modern hagfish in form. Sharks appeared about 450 mya, amphibians about 400 mya, reptiles about 350 mya, and mammals about 200 mya. No modern species should be described as more "primitive" than others, since all have an equally long evolutionary history, but the brains of modern hagfishes, lampreys, sharks, amphibians, reptiles, and mammals show a gradient of size and complexity that roughly follows the evolutionary sequence. All of these brains contain the same set of basic anatomical components, but many are rudimentary in hagfishes, whereas in mammals the foremost parts are greatly elaborated and expanded.
All vertebrate brains share a common underlying form, which can most easily be appreciated by examining how they develop. The first appearance of the nervous system is as a thin strip of tissue running along the back of the embryo. This strip thickens and then folds up to form a hollow tube. The front end of the tube develops into the brain. In its earliest form, the brain appears as three swellings, which eventually become the forebrain, midbrain, and hindbrain. In many classes of vertebrates these three parts remain similar in size in the adult, but in mammals the forebrain becomes much larger than the other parts, and the midbrain quite small.
Neuroanatomists usually consider the brain to consist of six main regions: the telencephalon (cerebral hemispheres), diencephalon (thalamus and hypothalamus), mesencephalon (midbrain), cerebellum, pons, and medulla. Each of these areas in turn has a complex internal structure. Some areas, such as the cortex and cerebellum, consist of layers, folded or convoluted to fit within the available space. Other areas consist of clusters of many small nuclei. If fine distinctions are made on the basis of neural structure, chemistry, and connectivity, thousands of distinguishable areas can be identified within the vertebrate brain.
Some branches of vertebrate evolution have led to substantial changes in brain shape, especially in the forebrain. The brain of a shark shows the basic components in a straightforward way, but in teleost fishes (the great majority of modern species), the forebrain has become "everted", like a sock turned inside out. In birds, also, there are major changes in shape. One of the main structures in the avian forebrain, the dorsal ventricular ridge, was long thought to correspond to the basal ganglia of mammals, but is now thought to be more closely related to the neocortex.

Several brain areas have maintained their identities across the whole range of vertebrates, from hagfishes to humans. Here is a list of some of the most important areas, along with a very brief description of their functions as currently understood (but note that the functions of most of them are still disputed to some degree):
- The medulla, along with the spinal cord, contains many small nuclei involved in a wide variety of sensory and motor functions.
- The hypothalamus is a small region at the base of the forebrain, whose complexity and importance belies its size. It is composed of numerous small nuclei, each with distinct connections and distinct neurochemistry. The hypothalamus is the central control station for sleep/wake cycles, control of eating and drinking, control of hormone release, and many other critical biological functions.
- Like the hypothalamus, the thalamus is a collection of nuclei with diverse functions. Some of them are involved in relaying information to and from the cerebral hemispheres. Others are involved in motivation. The subthalamic area (zona incerta) seems to contain action-generating systems for several types of "consummatory" behaviors, including eating, drinking, defecation, and copulation.
- The cerebellum modulates the outputs of other brain systems to make them more precise. Removal of the cerebellum does not prevent an animal from doing anything in particular, but it makes actions hesitant and clumsy. This precision is not built-in, but learned by trial and error. Learning how to ride a bicycle is an example of a type of neural plasticity that may take place largely within the cerebellum.
- The tectum, often called "optic tectum", allows actions to be directed toward points in space. In mammals it is called the "superior colliculus", and its best studied function is to direct eye movements. It also directs reaching movements, though. It gets strong visual inputs, but also inputs from other senses that are useful in directing actions, such as auditory input in owls, input from the thermosensitive pit organs in snakes, etc. In some fishes, such as lampreys, it is the largest part of the brain.
- The pallium is a layer of gray matter that lies on the surface of the forebrain. In reptiles and mammals it is called cortex instead. The pallium is involved in multiple functions, including olfaction and spatial memory. In mammals, where it comes to dominate the brain, it subsumes functions from many subcortical areas.
- The hippocampus, strictly speaking, is found only in mammals. However, the area it derives from, the medial pallium, has counterparts in all vertebrates. There is evidence that this part of the brain is involved in spatial memory and navigation in fishes, birds, reptiles, and mammals.
- The basal ganglia are a group of interconnected structures in the forebrain, of which our understanding has increased enormously over the last few years. The primary function of the basal ganglia seems to be action selection. They send inhibitory signals to all parts of the brain that can generate actions, and in the right circumstances can release the inhbition, so that the action-generating systems are able to execute their actions. Rewards and punishments exert their most important neural effects within the basal ganglia.
- The olfactory bulb is a special structure that processes olfactory sensory signals, and sends its output to the olfactory part of the pallium. It is a major brain component in many vertebrates, but much reduced in primates.
Mammals
The hindbrain and midbrain of mammals are generally similar to those of other vertebrates, but dramatic differences appear in the forebrain, which is not only greatly enlarged, but also altered in structure. In mammals, the surface of the cerebral hemispheres is mostly covered with 6-layered isocortex, more complex than the 3-layered pallium (neuroanatomy) seen in most vertebrates. Also the hippocampus of mammals has a distinctive structure.
Unfortunately, the evolutionary history of these mammalian features, especially the 6-layered cortex, is difficult to work out. This is largely because of a missing link problem. The ancestors of mammals, called synapsids, split off from the ancestors of modern reptiles and birds about 350 million years ago. However, the most recent branching that has left living results within the mammals was the split between monotremes (the platypus and echidna), marsupials (opossum, kangaroo, etc.) and placentals (most living mammals), which took place about 120 million years ago. The brains of monotremes and marsupials are distinctive from those of placentals in some ways, but they have fully mammalian cortical and hippocampal structures. Thus, these structures must have evolved between 350 and 120 million years ago, a period that has left no evidence except fossils, which do not preserve tissue as soft as brain.
Primates, including humans
See also: Human brainThe primate brain contains the same structures as the brains of other mammals, but is considerably larger in proportion to body size. Most of the enlargement comes from a massive expansion of the cortex, focusing especially on the parts subserving vision and forethought. The visual processing network of primates is very complex, including at least 30 distinguishable areas, with a bewildering web of interconnections. Taking all of these together, visual processing makes use of about half of the brain. The other part of the brain that is greatly enlarged is the prefrontal cortex, whose functions are difficult to summarize succinctly, but relate to planning, working memory, motivation, attention, and executive control.
Microscopic structure

Ranvier Axon terminal Schwann cell Myelin sheathNeuron
The brain is composed of two broad classes of cells: neurons and glia. These two types are equally numerous in the brain as a whole, although glial cells outnumber neurons roughly 4 to 1 in the cerebral cortex. Glia come in several types, which perform a number of critical functions, including structural support, metabolic support, insulation, and guidance of development.
The property that makes neurons so important is that, unlike glia, they are capable of sending signals to each other over long distances. They send these signals by means of an axon, a thin protoplasmic fiber that extends from the cell body and projects, usually with numerous branches, to other areas, sometimes nearby, sometimes in distant parts of the brain or body. The extent of an axon can be extraordinary: to take an example, if a pyramidal cell of the neocortex were magnified so that its cell body became the size of a human, its axon, equally magnified, would become a cable a few centimeters in diameter, extending farther than a kilometer. These axons transmit signals in the form of electrochemical pulses called action potentials, lasting less than a thousandth of a second and traveling along the axon at speeds of 1–100 meters per second. Some neurons emit action potentials constantly, at rates of 10–100 per second, usually in irregular temporal patterns; other neurons are quiet most of the time, but occasionally emit a burst of action potentials.
Axons transmit signals to other neurons, or to non-neuronal cells, by means of specialized junctions called synapses. A single axon may make as many as several thousand synaptic connections. When an action potential, traveling along an axon, arrives at a synapse, it causes a chemical called a neurotransmitter to be released. The neurotransmitter binds to receptor molecules in the membrane of the target cell. Some types of neuronal receptors are excitatory, meaning that they increase the rate of action potentials in the target cell; other receptors are inhibitory, meaning that they decrease the rate of action potentials; others have complex modulatory effects on the target cell.
Axons actually fill most of the space in the brain. Often large groups of them are bundles together in what are called nerve fiber tracts. In many cases, each axon is wrapped in a thick sheath of a fatty substance called myelin, which serves to greatly increase the speed of action potential propagation. Myelin is white in color, so parts of the brain filled exclusively with nerve fibers appear as white matter, in contrast to the gray matter that marks areas where high densities of neuron cell bodies are located. The total length of these myelinated axons in the human brain is considerable: 176,000 kilometres (109,000 mi) in a 20-year-old male and 149,000 kilometres (93,000 mi) in a female.
Development

The brain does not simply grow; it develops in an intricately orchestrated sequence of steps. Many neurons are created in special zones that contain stem cells, and then migrate through the tissue to reach their ultimate locations. In the cortex, for example, the first stage of development is the formation of a "scaffold" by a special group of glial cells, called radial glia, which send fibers vertically across the cortex. New cortical neurons are created at the bottom of the cortex, and then "climb" along the radial fibers until they reach the layers they are destined to occupy in the adult.
Once a neuron is in place, it begins to extend dendrites and an axon into the area around it. Axons, because they commonly extend a great distance from the cell body and need to make contact with specific targets, grow in a particularly complex way. The tip of a growing axon consists of a blob of protoplasm called a "growth cone", studded with chemical receptors. These receptors sense the local environment, causing the growth cone to be attracted or repelled by various cellular elements, and thus to be pulled in a particular direction at each point along its path. The result of this pathfinding process is that the growth cone navigates through the brain until it reaches its destination area, where other chemical cues cause it to begin generating synapses. Taking the entire brain into account, many thousands of genes give rise to proteins that influence axonal pathfinding.
The synaptic network that finally emerges is only partly determined by genes, though. In many parts of the brain, axons initially "overgrow", and then are "pruned" by mechanisms that depend on neural activity. In the projection from the eye to the midbrain, for example, the structure in the adult contains a very precise mapping, connecting each point on the surface of the retina to a corresponding point in a midbrain layer. In the first stages of development, each axon from the retina is guided to the right general vicinity in the midbrain by chemical cues, but then branches very profusely and makes initial contact with a wide swath of midbrain neurons. The retina, before birth, contains special mechanisms that cause it to generate waves of activity that originate spontaneously at some point and then propagate slowly across the retinal layer. These waves are useful because they cause neighboring neurons to be active at the same time: that is, they produce a neural activity pattern that contains information about the spatial arrangement of the neurons. This information is exploited in the midbrain by a mechanism that causes synapses to weaken, and eventually vanish, if activity in an axon is not followed by activity of the target cell. The result of this sophisticated process is a gradual tuning and tightening of the map, leaving it finally in its precise adult form.
Similar things happen in other brain areas: an initial synaptic matrix is generated as a result of genetically determined chemical guidance, but then gradually refined by activity-dependent mechanisms, partly driven by internal dynamics, partly by external sensory inputs. In some cases, as with the retina-midbrain system, activity patterns depend on mechanisms that operate only in the developing brain, and apparently exist solely for the purpose of guiding development.
In humans and many other mammals, new neurons are created mainly before birth, and the infant brain actually contains substantially more neurons than the adult brain. There are, however, a few areas where new neurons continue to be generated throughout life. The two areas for which this is well established are the olfactory bulb, which is involved in the sense of smell, and the dentate gyrus of the hippocampus, where there is evidence that the new neurons play a role in storing newly acquired memories. With these exceptions, however, the set of neurons that is present in early childhood is the set that is present for life. Glial cells are different, however; as with most types of cells in the body, these are generated throughout the lifespan.
Although the pool of neurons is largely in place by birth, the axonal connections continue to develop for a long time afterward. In humans, full myelination is not completed until adolescence.
There has long been debate about whether the qualities of mind, personality, and intelligence can mainly be attributed to heredity or to upbringing; the nature versus nurture debate. This is not just a philosophical question: it has great practical relevance to parents and educators. Although many details remain to be settled, neuroscience clearly shows that both factors are essential. Genes determine the general form of the brain, and genes determine how the brain reacts to experience. Experience, however, is required to refine the matrix of synaptic connections. In some respects it is mainly a matter of presence or absence of experience during critical periods of development. In other respects, the quantity and quality of experience may be more relevant: for example, there is substantial evidence that animals raised in enriched environments have thicker cortices, indicating a higher density of synaptic connections, than animals whose levels of stimulation are restricted.
Functions
From a biological perspective, the function of a brain is to generate behaviors that promote the genetic fitness of an animal. To do this, it extracts enough relevant information from sense organs to refine actions. Sensory signals may stimulate an immediate response as when the olfactory system of a deer detects the odor of a wolf; they may modulate an ongoing pattern of activity as in the effect of light-dark cycles on an organism's sleep-wake behavior; or their information may be stored in case of future relevance. The brain manages its complex task by orchestrating functional subsystems, which can be categorized in a number of ways: anatomically, chemically, and functionally.
Neurotransmitter systems
Main article: Neurotransmitter systemsWith few exceptions, each neuron in the brain consistently releases the same chemical neurotransmitter, or set of neurotransmitters, at all of the synaptic connections it makes with other neurons. Thus, a neuron can be characterized by the neurotransmitters it releases. The two neurotransmitters that appear most frequently are glutamate, which is almost always excitatory, and gamma-aminobutyric acid (GABA), which is almost always inhibitory. Neurons using these transmitters can be found in nearly every part of the brain, making up a large percentage of the brain's pool of synapses.
Nevertheless, the great majority of psychoactive drugs exert their effects by altering neurotransmitter systems not directly involving glutamatergic or GABAergic transmission. Drugs such as caffeine, nicotine, heroin, cocaine, Prozac, Thorazine, etc., act on other neurotransmitters. Many of these other transmitters come from neurons that are localized in particular parts of the brain. Serotonin, for example—the primary target of antidepressant drugs and many dietary aids—comes exclusively from a small brainstem area called the Raphe nuclei. Norepinephrine, which is involved in arousal, comes exclusively from a nearby small area called the locus ceruleus. Histamine, as a neurotransmitter, comes from a tiny part of the hypothalamus called the tuberomammilary nucleus (histamine also has non-CNS functions, but the neurotransmitter function is what causes antihistamines to have sedative effects). Other neurotransmitters such as acetylcholine and dopamine have multiple sources in the brain, but are not as ubiquitously distributed as glutamate and GABA.
Sensory systems
Main article: Sensory systemOne of the primary functions of a brain is to extract biologically relevant information from sensory inputs. Even in the human brain, sensory processes go well beyond the classical five senses of sight, sound, taste, touch, and smell: our brains are provided with information about temperature, balance, limb position, and the chemical composition of the bloodstream, among other things. All of these modalities are detected by specialized sensors that project signals into the brain. In other animals, additional senses may be present, such as the infrared heat-sensors in the pit organs of snakes; or the "standard" senses may be used in nonstandard ways, as in the auditory "sonar" of bats. Here are a few principles that apply to most sensory systems, using the sense of hearing for specific examples:
- Each system begins with specialized "sensory receptor" cells. These are neurons, but unlike most neurons, they are not controlled by synaptic input from other neurons: instead they are activated by membrane-bound receptors that are sensitive to some physical modality, such as light, temperature, or physical stretching. The axons of sensory receptor cells travel into the spinal cord or brain. For the sense of hearing, the receptors are located in the inner ear, on the cochlea, and are activated by vibration.
- For most senses, there is a "primary nucleus" or set of nuclei, located in the brainstem, that gathers signals from the sensory receptor cells. For the sense of hearing, these are the cochlear nuclei.
- In many cases, there are secondary subcortical areas that extract special information of some sort. For the sense of hearing, the superior olivary area and inferior colliculus are involved in comparing the signals from the two ears to extract information about the direction of the sound source, among other functions.
- Each sensory system also has a special part of the thalamus dedicated to it, which serves as a relay to the cortex. For the sense of hearing, this is the medial geniculate nucleus.
- For each sensory system, there is a "primary" cortical area that receives direct input from the thalamic relay area. For the auditory system this is the primary auditory cortex, located in the upper part of the temporal lobe.
- There are also usually a set of "higher level" cortical sensory areas, which analyze the sensory input in specific ways. For the auditory system, there are areas that analyze sound quality, rhythm, and temporal patterns of change, among other features.
- Finally, there are multimodal areas that combine inputs from different sensory modalities, for example auditory and visual. At this point, the signals have reached parts of the brain that are best described as integrative rather than specifically sensory.
All of these rules have exceptions. For example, the sense of touch (which is actually a set of at least half-a-dozen distinct mechanical senses), the sensory inputs terminate mainly in the spinal cord, on neurons that then project to the brainstem. For the sense of smell, there is no relay in the thalamus; instead the signals go directly from the primary brain area—the olfactory bulb—to the cortex.
Motor system
Main article: Motor systemMotor systems are areas of the brain that are more or less directly involved in producing body movements, that is, in activating muscles. With the exception of the muscles that control the eye, all of the voluntary muscles in the body are directly innervated by motor neurons in the spinal cord, which therefore are the final common path for the movement-generating system. Spinal motor neurons are controlled both by neural circuits intrinsic to the spinal cord, and by inputs that descend from the brain. The intrinsic spinal circuits implement many reflex responses, and also contain pattern generators for rhythmic movements such as walking or swimming. The descending connections from the brain allow for more sophisticated control.
The brain contains a number of areas that project directly to the spinal cord. At the lowest level are motor areas in the medulla and pons. At a higher level are areas in the midbrain, such as the red nucleus, which is responsible for coordinating movements of the arms and legs. At a higher level yet is the primary motor cortex, a strip of tissue located at the posterior edge of the frontal lobe. The primary motor cortex sends projections to the subcortical motor areas, but also sends a massive projection directly to the spinal cord, via the so-called pyramidal tract. This direct corticospinal projection allows for precise voluntary control of the fine details of movements. Other "secondary" motor-related brain areas do not project directly to the spinal cord, but instead act on the cortical or subcortical primary motor areas. Among the most important secondary areas are the premotor cortex, basal ganglia, and cerebellum:
- The premotor cortex (which is actually a large complex of areas) adjoins the primary motor cortex, and projects to it. Whereas elements of the primary motor cortex map to specific body areas, elements of the premotor cortex are often involved in coordinated movements of multiple body parts.
- The basal ganglia are a set of structures in the base of the forebrain that project to many other motor-related areas. Their function has been difficult to understand, but one of the most popular theories currently is that they play a key role in action selection. Most of the time they restrain actions by sending constant inhibitory signals to action-generating systems, but in the right circumstances, they release this inhibition and therefore allow their targets to take control of behavior.
- The cerebellum is a very distinctive structure attached to the back of the brain. It does not control or originate behaviors, but instead generates corrective signals to make movements more precise. People with cerebellar damage are not paralyzed in any way, but their body movements become erratic and uncoordinated.
In addition to all of the above, the brain and spinal cord contain extensive circuitry to control the autonomic nervous system, which works by secreting hormones and by modulating the "smooth" muscles of the gut. The autonomic nervous system affects heart rate, digestion, respiration rate, salivation, perspiration, urination, and sexual arousal—but most of its functions are not under direct voluntary control.
Arousal system
See also: SleepPerhaps the most obvious aspect of the behavior of any animal is the daily cycle between sleeping and waking. Arousal and alertness are also modulated on a finer time scale, though, by an extensive network of brain areas.
A key component of the arousal system is the suprachiasmatic nucleus (SCN), a tiny part of the hypothalamus located directly above the point at which the optic nerves from the two eyes cross. The SCN contains the body's central biological clock. Neurons there show activity levels that rise and fall with a period of about 24 hours, circadian rhythms: these activity fluctuations are driven by rhythmic changes in expression of a set of "clock genes". The SCN continues to keep time even if it is excised from the brain and placed in a dish of warm nutrient solution, but it ordinarily receives input from the optic nerves, through the retinohypothalamic tract (RHT), that allow daily light-dark cycles to calibrate the clock.
The SCN projects to a set of areas in the hypothalamus, brainstem, and midbrain that are involved in implementing sleep-wake cycles. An important component of the system is the so-called reticular formation, a group of neuron-clusters scattered diffusely through the core of the lower brain. Reticular neurons send signals to the thalamus, which in turn sends activity-level-controlling signals to every part of the cortex. Damage to the reticular formation can produce a permanent state of coma.
Sleep involves great changes in brain activity. Until the 1950s it was generally believed that the brain essentially shuts off during sleep, but this is now known to be far from true: activity continues, but patterns become very different. In fact, there are two types of sleep, REM sleep (with dreaming) and NREM (non-REM, usually without dreaming) sleep, which repeat in slightly varying patterns throughout a sleep episode. Three broad types of distinct brain activity patterns can be measured: REM, light NREM and deep NREM. During deep NREM sleep, also called slow wave sleep, activity in the cortex takes the form of large synchronized waves, where in the waking state it is noisy and desynchronized. Levels of the neurotransmitters norepinephrine and serotonin drop during slow wave sleep, and fall almost to zero during REM sleep; levels of acetylcholine show the reverse pattern.
Brain energy consumption

Although the human brain represents only 2% of the body weight, it receives 15% of the cardiac output, 20% of total body oxygen consumption, and 25% of total body glucose utilization. The need to limit body weight in order, for example, to fly, has led to selection for a reduction of brain size in some species, such as bats. The brain mostly utilizes glucose for energy, and deprivation of glucose, as can happen in hypoglycemia, can result in loss of consciousness. The energy consumption of the brain does not vary greatly over time, but active regions of the cortex consume somewhat more energy than inactive regions: this fact forms the basis for the functional brain imaging methods PET and fMRI. These are nuclear medicine imaging techniques which produce a three-dimensional image of metabolic activity.
Brain and mind
See also: Philosophies of mindUnderstanding the relationship between the brain and the mind is a challenging problem both philosophically and scientifically. The most straightforward scientific evidence that there is a strong relationship between the physical brain matter and the mind is the impact physical alterations to the brain have on the mind, such as with traumatic brain injury and psychoactive drug use.
The mind-body problem is one of the central issues in the history of philosophy, which asks us to consider if the brain and the mind are identical, partially distinct, or related in some unknown way. There are three major schools of thought concerning the answer: dualism, materialism, and idealism. Dualism holds that the mind exists independently of the brain; materialism holds that mental phenomena are identical to neuronal phenomena; and idealism holds that only mental phenomena exist. In addition to the philosophical questions, the relationship between mind and brain involves a number of scientific questions, including understanding the relationship between mental activity and brain activity, the exact mechanisms by which drugs influence cognition, and the neural correlates of consciousness.
Through most of history many philosophers found it inconceivable that cognition could be implemented by a physical substance such as brain tissue. Philosophers such as Patricia Churchland posit that the drug-mind interaction is indicative of an intimate connection between the brain and the mind, not that the two are the same entity. Even Descartes, notable for his mechanistic philosophy, who found it possible to explain reflexes and other simple behaviors in mechanistic terms, could not believe that complex thought, language in particular, could be explained by reference to the physical brain alone.
How it is studied
Main article: NeuroscienceNeuroscience seeks to understand the nervous system, including the brain, from a biological and computational perspective. Psychology seeks to understand mind and behavior. Neurology is the medical discipline that diagnoses and treats pathologies of the nervous system. The brain is also the most important organ studied in psychiatry, the branch of medicine that works to study, prevent, and treat mental disorders. Cognitive science seeks to unify neuroscience and psychology with other fields that concern themselves with the brain, such as computer science (artificial intelligence and similar fields) and philosophy. Some methods of examining the brain are mainly useful in humans, and are described in the human brain article. This section focuses on methods that are usable across a wide range of animal species, though the great majority of basic neuroscience experiments are done using rats or mice as subjects.
Neuroanatomy
Main article: NeuroanatomyThe oldest method of studying the brain is anatomical, and until the middle of the 20th century, much of the progress in neuroscience came from the development of better stains and better microscopes—the neuroanatomist Floyd Bloom famously quipped that "the gain in brain is mainly in the stain." Later, much critical information about synaptic function came from study of electron microscope images of synapses. On a larger scale, neuroanatomists have invented a plethora of stains that reveal neural structure, chemistry, and connectivity. In recent years, the development of immunostaining techniques has allowed staining of neurons that express specific sets of genes.
Electrophysiology
Electrophysiology allows scientists to record the electrical activity of individual neurons or groups of neurons. There are two general approaches: intracellular and extracellular recordings.
Intracellular recording uses glass electrodes with very fine tips in order to pick up electrical signals from the interior of a neuron. This method is very sensitive, but also very delicate, and is usually carried out in vitro—i.e., in a dish of warm nutrient solution; using tissue that has been extracted from the brain of an animal. Because the tissue is irreversibly damaged in the process, the method is invasive.
Extracellular recording uses larger electrodes that can be used in the brains of living animals. This method cannot usually resolve the tiny electrical signals generated by individual synaptic connections, but it can pick up action potentials generated by individual neurons, as well as field potentials generated by synchronous synaptic activity in large groups of neurons. Because the brain does not contain pain receptors, it is possible using these techniques to record from animals that are awake and behaving without causing distress. The same techniques have occasionally been used to study brain activity in human patients suffering from intractable epilepsy, in cases where there was a medical necessity to implant electrodes in order to localize the brain area responsible for seizures.
Lesion studies
See also: Neurology and Brain damageEven though it is protected by the skull and meninges, surrounded by cerebrospinal fluid, and isolated from the bloodstream by the blood-brain barrier, the delicate nature of the brain makes it vulnerable to numerous diseases and several types of damage. In humans, the effects of strokes and other types of brain damage have been a key source of information about brain function. Because there is no ability to experimentally control the nature of the damage, however, this information is often difficult to interpret. In animal studies, most commonly involving rats, it is possible to use electrodes or locally injected chemicals to produce precise patterns of damage and then examine the consequences for behavior.
Computation
Main article: Computational neuroscienceA computer, in the broadest sense, is a device for storing and processing information. In an ordinary digital computer, information is represented by electronic circuits that have two stable states, often denoted 0 and 1. In a brain, information is represented both dynamically, by trains of action potentials in neurons, and statically, by the strengths of synaptic connections between neurons. In a digital computer, information is processed by a small set of "registers" that operate at speeds of billions of cycles per second. In a brain, information is processed by billions of neurons all operating simultaneously, but only at speeds around 100 cycles per second. Thus, brains and digital computers are similar in that both are devices for processing information, but the ways that they do it are very different.
Computational neuroscience encompasses two approaches: first, the use of computers to study the brain; second, the study of how brains perform computation. On one hand, it is possible to write a computer program to simulate the operation of a group of neurons by making use of systems of equations that describe their electrochemical activity; such simulations are known as biologically realistic neural networks. On the other hand, it is possible to study algorithms for neural computation by simulating, or mathematically analyzing, the operations of simplified "units" that have some of the properties of neurons but abstract out much of their biological complexity.
Most programs for digital computers rely on long sequences of operations executed in a specific order, and therefore could not be "ported" into a brain without becoming extremely slow. Computer scientists, however, have found that some types of problems lend themselves naturally to algorithms that can efficiently be executed by brainlike networks of processing elements. One important problem that falls into this group is object recognition: on a digital computer, the seemingly simple task of recognizing a face in a photo turns out to be tremendously difficult, and even the best current programs don't do it well; the human brain, however, reliably solves this problem in a fraction of a second. The process feels almost effortless, but this is only because our brains are heavily optimized for it. Other tasks that are computationally a great deal simpler, such as adding pairs of hundred-digit numbers, feel more difficult because the human brain is not adapted to execute them efficiently.
The computational functions of the brain are studied both by neuroscientists and computer scientists. There have been several attempts to build electronic computers that operate on brainlike principles, including a supercomputer called the Connection Machine, but to date none of them has achieved notable success. Brains have several advantages that are difficult to duplicate in an electronic device, including (1) the microscopic size of the processing elements, (2) the three-dimensional arrangement of connections, and (3) the fact that each neuron generates its own power (metabolically).
Genetics
Recent years have seen the first applications of genetic engineering techniques to the study of the brain. The most common subjects are mice, because the technical tools are more advanced for this species than for any other. It is now possible with relative ease to "knock out" or mutate a wide variety of genes, and then examine the effects on brain function. More sophisticated approaches are also beginning to be used: for example, using the Cre-Lox recombination method it is possible to activate or inactivate genes in specific parts of the brain, at specific times.
History of its study
See also: History of the brainEarly views were divided as to whether the seat of the soul lies in the brain or heart. On one hand, it was impossible to miss the fact that awareness feels like it is localized in the head, and that blows to the head can cause unconsciousness much more easily than blows to the chest, and that shaking the head causes dizziness. On the other hand, the brain to a superficial examination seems inert, whereas the heart is constantly beating. Cessation of the heartbeat means death; strong emotions produce changes in the heartbeat; and emotional distress often produces a sensation of pain in the region of the heart ("heartache"). Aristotle favored the heart, and thought that the function of the brain was merely to cool the blood. Democritus, the inventor of the atomic theory of matter, favored a three-part soul, with intellect in the head, emotion in the heart, and lust in the vicinity of the liver. Hippocrates, the "father of medicine", was entirely in favor of the brain. In On the Sacred Disease, his account of epilepsy, he wrote:
Men ought to know that from nothing else but the brain come joys, delights, laughter and sports, and sorrows, griefs, despondency, and lamentations. ... And by the same organ we become mad and delirious, and fears and terrors assail us, some by night, and some by day, and dreams and untimely wanderings, and cares that are not suitable, and ignorance of present circumstances, desuetude, and unskilfulness. All these things we endure from the brain, when it is not healthy...
—Hippocrates, On the Sacred Disease
The famous Roman physician Galen also advocated the importance of the brain, and theorized in some depth about how it might work. Even after physicians and philosophers had accepted the primacy of the brain, though, the idea of the heart as seat of intelligence continued to survive in popular idioms, such as "learning something by heart". Galen did a masterful job of tracing out the anatomical relationships between brain, nerves, and muscles, demonstrating that all muscles in the body are connected to the brain via a branching network of nerves. He postulated that nerves activate muscles mechanically, by carrying a mysterious substance he called pneumata psychikon, usually translated as "animal spirits". His ideas were widely known during the Middle Ages, but not much further progress came until the Renaissance, when detailed anatomical study resumed, combined with the theoretical speculations of Descartes and his followers. Descartes, like Galen, thought of the nervous system in hydraulic terms. He believed that the highest cognitive functions—language in particular—are carried out by a non-physical res cogitans, but that the majority of behaviors of humans and animals could be explained mechanically. The first real progress toward a modern understanding of nervous function, though, came from the investigations of Luigi Galvani, who discovered that a shock of static electricity applied to an exposed nerve of a dead frog could cause its leg to contract.

Each major advance in understanding has followed more or less directly from the development of a new method of investigation. Until the early years of the 20th century, the most important advances were derived from new stains. Particularly critical was the invention of the Golgi stain, which (when correctly used) stains only a small, and apparently random, fraction of neurons, but stains them in their entirety, including cell body, dendrites, and axon. Without such a stain, brain tissue under a microscope appears as an impenetrable tangle of protoplasmic fibers, in which it is impossible to determine any structure. In the hands of Camillo Golgi, and especially of the Spanish neuroanatomist Santiago Ramon y Cajal, the new stain revealed hundreds of distinct types of neurons, each with its own unique dendritic structure and pattern of connectivity.
In the 20th century, progress in electronics enabled investigation of the electrical properties of nerve cells, culminating in the work by Alan Hodgkin, Andrew Huxley, and others on the biophysics of the action potential, and the work of Bernard Katz and others on the electrochemistry of the synapse. The earliest studies used special preparations, such as the "fast escape response" system of the squid, which involves a giant axon as thick as a pencil lead, and giant synapses connecting to this axon. Steady improvements in electrodes and electronics allowed ever finer levels of resolution. These studies complemented the anatomical picture with a conception of the brain as a dynamic entity. Reflecting the new understanding, in 1942 Charles Sherrington visualized the workings of the brain in action in somewhat breathless terms:
The great topmost sheet of the mass, that where hardly a light had twinkled or moved, becomes now a sparkling field of rhythmic flashing points with trains of traveling sparks hurrying hither and thither. ... It is as if the Milky Way entered upon some cosmic dance. Swiftly the head mass becomes an enchanted loom where millions of flashing shuttles weave a dissolving pattern, always a meaningful pattern though never an abiding one; a shifting harmony of subpatterns.
—Sherrington, 1942, Man on his Nature
The 1990s were known in the US as the "Decade of the Brain" to commemorate advances made in brain research, and to promote funding for such research.
See also
Notes
- ^ Shepherd, Neurobiology
- Pelvig et al., 2008
- AlonsoNanclares et al., 2008
- Gehring, 2005
- Nickel, 2002
- Grillner & Wallén, 2002
- Sejnowski, 23 Problems in Systems Neuroscience
- Shepherd, Neurobiology, p 3
- Aboitiz et al., 2003
- Armstrong, 1983
- Jerison, Evolution of the Brain and Intelligence
- ^ Finlay et al., 2001
- ^ Balavoine & Adoutte, 2003
- Schmidt-Rhaesa, Evolution of Organ Systems, p 110
- ^ Butler, 2000
- Kandel, In Search of Memory
- Flybrain web site
- Konopka & Benzer, 1971
- Shin et al., 1985
- WormBook web site
- Hobert, WormBook
- White et al., 1986
- Principles of neural science, Ch. 17
- Carpenter's Human Neuroanatomy, Ch. 1
- Shu et al., 2003
- Striedter, Principles of Brain Evolution
- Principles of Neural Science, p 1019
- Principles of Neural Science, Ch. 17
- Northcutt, 2008
- Reiner et al., 2005
- Principles of Neural Science, Ch. 44, 45
- Swaab et al., The Human Hypothalamus
- Jones, The Thalamus
- ^ Principles of Neural Science, Ch. 42
- Saitoh et al., 2007
- Puelles, 2001
- Salas et al., 2003
- Grillner et al., 2005
- Northcutt, 1981
- Barton & Harvey, 2000
- Aboitiz, 2004
- Calvin, How Brains Think
- Principles of Neural Science p 20
- Azevedo, FA (2009). "Equal numbers of neuronal and nonneuronal cells make the human brain an isometrically scaled-up primate brain". J Comp Neurol. 5 (513): 532–41. PMID 19226510.
{{cite journal}}
: Unknown parameter|coauthors=
ignored (|author=
suggested) (help) - Principles of Neural Science, p 21
- Principles of Neural Science, Ch. 10
- Principles of Neural Science, Ch. 2
- Marner, L (2003). "Marked loss of myelinated nerve fibers in the human brain with age". J Comp Neurol. 2 (462): 144–52. PMID 12794739.
{{cite journal}}
: Unknown parameter|coauthors=
ignored (|author=
suggested) (help) - Principles of Neural Development, Ch. 1
- Principles of Neural Development, Ch. 4
- Principles of Neural Development, Chs. 5, 7
- Principles of Neural Development, Ch. 12
- ^ Wong, 1999
- Principles of Neural Development, Ch. 6
- Paus et al., 2001
- Ridley, Nature vs Nurture
- Wiesel, 1982
- van Praag et al., 2000
- Carew, Behavioral Neurobiology, Ch. 1
- Principles of Neural Science, Ch. 15
- P.L. McGeer and E.G. McGeer (1989) "Amino Acid Neurotransmitters" in Basic Neurochemistry, G. Siegel et al., eds. (New York: Raven Press), chapter 15, pp. 311–332.
- Cooper et al., Biochemical Basis of Neuropharmacology
- Principles of Neural Science, Ch. 21
- ^ Principles of Neural Science, Chs. 21, 30
- Principles of Neural Science, Ch. 23
- Principles of Neural Science, Ch. 32
- See muscle
- Principles of Neural Science, Ch. 34
- Principles of Neural Science, Chs. 36, 37
- Principles of Neural Science, Ch. 33
- Principles of Neural Science, Ch. 38
- Principles of Neural Science, Ch. 43
- Gurney et al., 2004
- Principles of Neural Science, Ch. 49
- ^ Principles of Neural Science, Ch. 45
- Antle & Silver, 2005
- Principles of Neural Science, Ch. 47
- Kleitman, Nathaniel (1938, revised 1963, reprinted 1987) Sleep and Wakefulness (Introduction). The University of Chicago Press, Midway Reprints series, ISBN 0-226-44073-7
- Clark & Sokoloff, 1999
- Safi et al., 2005
- Raichle & Gusnard, 2002
- Churchland, Neurophilosophy
- Boake C, Diller L (2005). "History of rehabilitation for traumatic brain injury". In High WM, Sander AM, Struchen MA, Hart KA (ed.). Rehabilitation for Traumatic Brain Injury. Oxford : Oxford University Press. ISBN 0-19-517355-4.
{{cite book}}
: CS1 maint: multiple names: editors list (link) - Albert, David Bruce, Jr. (1993). "Event Horizons of the Psyche".
{{cite web}}
: Unknown parameter|dateformat=
ignored (help)CS1 maint: multiple names: authors list (link) CS1 maint: numeric names: authors list (link) - Neurophilosophy, Ch. 7
- Hart, 1996
- ^ Lacey, 1996
- Neurophilosophy, Ch. 6
- Neurophilosophy, Ch. 8
- 1988. The Philosophical Writings Of Descartes in 3 vols. Cottingham, J., Stoothoff, R., Kenny, A., and Murdoch, D., trans. Cambridge University Press.
- Principles of Neural Science, Ch. 1
- Storrow, Outline of Clinical Psychiatry
- Neuroscience: Exploring the Brain, Ch. 2
- Dowling, Neurons and Networks, pages 15–24
- Dowling, Neurons and Networks, p. 22
- Wyllie et al., Treatment of Epilepsy, Ch. 77
- Fundamentals of Human Neuropsychology, Ch. 1
- ^ Abbott & Dayan, Theoretical Neuroscience
- von Neumann, 2000
- von Neumann, 2000, pp. 39–55
- Tonegawa et al., 2003
- Finger, Origins of Neuroscience, p 14
- Hippocrates, On the Sacred Disease
- Encyclopedia of Word and Phrase Origins
- Bloom, 1972, p 211
- Piccolino, 2002
- Sherrington, Man on his Nature
- Bush, George H.W. (1990). "Project on the Decade of the Brain". Retrieved 2009-09-30.
{{cite web}}
: Unknown parameter|month=
ignored (help)
References
- Abbott, LF (2001). Theoretical Neuroscience: Computational and Mathematical Modeling of Neural Systems. MIT Press. ISBN 9780262541855.
{{cite book}}
: Unknown parameter|coathors=
ignored (help) - Aboitiz, F (2003). "The evolutionary origin of the mammalian isocortex: Towards an integrated developmental and functional approach". Behav Brain Sci. 26: 535–52. doi:10.1017/S0140525X03000128. PMID 15179935.
{{cite journal}}
: Unknown parameter|coauthors=
ignored (|author=
suggested) (help) - Alonso-Nanclares L, Gonzalez-Soriano J, Rodriguez JR, DeFelipe J (2008). "Gender differences in human cortical synaptic density". Proc Nat Acad Sci. 105: 14615–9. PMID 18779570.
{{cite journal}}
: Unknown parameter|pmcid=
ignored (|pmc=
suggested) (help)CS1 maint: multiple names: authors list (link) - Antle, MC (2005). "Orchestrating time: arrangements of the brain circadian clock" (PDF). Trends Neurosci. 28: 145–51. doi:10.1016/j.tins.2005.01.003. PMID 15749168.
{{cite journal}}
: Unknown parameter|coauthors=
ignored (|author=
suggested) (help) - Armstrong, E (1983). "Relative brain size and metabolism in mammals". Science. 220: 1302–4. doi:10.1126/science.6407108. PMID 6407108.
- Balavoine, G (2003). "The segmented Urbilateria: A testable scenario". Int Comp Biology. 43: 137–47. doi:10.1093/icb/43.1.137.
{{cite journal}}
: Unknown parameter|coauthors=
ignored (|author=
suggested) (help) - Barton, RA (2000). "Mosaic evolution of brain structure in mammals". Nature. 405: 1055–8. doi:10.1038/35016580. PMID 10890446.
{{cite journal}}
: Unknown parameter|coauthors=
ignored (|author=
suggested) (help) - Bear, MF (2007). Neuroscience: Exploring the Brain. Lippincott Williams & Wilkins. ISBN 9780781760034.
{{cite book}}
: Unknown parameter|coauthors=
ignored (|author=
suggested) (help) - Bloom, FE (1975). The Neurosciences, Paths of Discovery. MIT Press. ISBN 9780262230728.
{{cite book}}
: Unknown parameter|editors=
ignored (|editor=
suggested) (help) - Butler, AB (2000). "Chordate Evolution and the Origin of Craniates: An Old Brain in a New Head". Anat Rec. 261: 111–25. doi:10.1002/1097-0185(20000615)261:3<111::AID-AR6>3.0.CO;2-F. PMID 10867629.
- Calvin, WH (1996). How Brains Think. Basic Books. ISBN 9780465072781.
- Carew, TJ (2000). Behavioral Neurobiology: the Cellular Organization of Natural Behavior. Sinauer Associates. ISBN 9780878930920.
- Churchland, PS (1989). Neurophilosophy. MIT Press. ISBN 9780262530859.
- Clark, DD (1999). Siegel GJ, Agranoff BW, Albers RW, Fisher SK, Uhler MD (ed.). Basic Neurochemistry: Molecular, Cellular and Medical Aspects. Philadelphia: Lippincott. pp. 637–70. ISBN 9780397518203.
{{cite book}}
: Unknown parameter|coauthors=
ignored (|author=
suggested) (help)CS1 maint: multiple names: editors list (link) - Cooper, JR (2003). The Biochemical Basis of Neuropharmacology. Oxford University Press US. ISBN 9780195140088.
{{cite book}}
: Unknown parameter|coauthors=
ignored (|author=
suggested) (help) - Dowling, JE (2001). Neurons and Networks. Harvard University Press. ISBN 9780674004627.
- Finger, S (2001). Origins of Neuroscience. Oxford University Press. ISBN 9780195146943.
- Finlay, BL (2001). "Developmental structure in brain evolution". Behav Brain Sci. 20: 263–308. PMID 11530543.
{{cite journal}}
:|format=
requires|url=
(help); Unknown parameter|coauthors=
ignored (|author=
suggested) (help) - Gehring, WJ (2005). "New Perspectives on Eye Development and the Evolution of Eyes and Photoreceptors: The Evolution of Eyes and Brain" (Full text). J Heredity. 96: 171–184. doi:10.1093/jhered/esi027. PMID 15653558. Retrieved 2008-04-26.
- Grillner, S (2005). "Mechanisms for selection of basic motor programs—roles for the striatum and pallidum". Trends Neurosci. 28: 364–70. doi:10.1016/j.tins.2005.05.004. PMID 15935487.
{{cite journal}}
: Unknown parameter|coauthors=
ignored (|author=
suggested) (help) - Grillner, S (2002). "Cellular bases of a vertebrate locomotor system-steering, intersegmental and segmental co-ordination and sensory control". Brain Res Brain Res Rev. 40: 92–106. doi:10.1016/S0165-0173(02)00193-5. PMID 12589909.
{{cite journal}}
: Unknown parameter|coauthors=
ignored (|author=
suggested) (help) - Gurney, K (2004). "Computational models of the basal ganglia: from robots to membranes". Trends Neurosci. 27: 453–459. doi:10.1016/j.tins.2004.06.003. PMID 15271492.
{{cite journal}}
: Unknown parameter|coauthors=
ignored (|author=
suggested) (help) - Hart, WD (1996). Guttenplan S (ed.). A Companion to the Philosophy of Mind. Blackwell. pp. 265–7.
- Hendrickson, R (2000). The Facts on File Encyclopedia of Word and Phrase Origins. New York: Facts on File. ISBN 978-0816040889.
- Hippocrates (400 B.C.E.). On the Sacred Disease. Translated by Francis Adams.
{{cite book}}
: Check date values in:|year=
(help)CS1 maint: year (link) - Hobert, O (2005), The C. elegans Research Community (ed.), "Wormbook", WormBook, doi:10.1895/wormbook.1.12.1
{{citation}}
:|contribution=
ignored (help) - Jerison, HJ (1973). Evolution of the Brain and Intelligence. Academic Press. ISBN 9780123852502.
- Jones, EG (1985). The Thalamus. Plenum Press. ISBN 9780306418563.
- Kandel, ER (2000). Principles of Neural Science. McGraw-Hill Professional. ISBN 9780838577011.
{{cite book}}
: Unknown parameter|coauthors=
ignored (|author=
suggested) (help) - Kandel, ER (2007). In Search of Memory: The Emergence of a New Science of Mind. WW Norton. ISBN 9780393329377.
- Kolb, B (2008). Fundamentals of Human Neuropsychology. Macmillan. ISBN 9780716795865.
{{cite book}}
: Unknown parameter|coauthors=
ignored (|author=
suggested) (help) - Konopka, RJ (1971). "Clock mutants of Drosophila melanogaster". PNAS. 68: 2112–6. doi:10.1073/pnas.68.9.2112. PMID 5002428.
{{cite journal}}
: Unknown parameter|coauthors=
ignored (|author=
suggested) (help); Unknown parameter|pmcid=
ignored (|pmc=
suggested) (help) - Lacey, A (1996). A Dictionary of Philosophy. Routledge.
- Nickel, M (2002). "Dynamics and cellular movements in the locomotion of the sponge Tethya wilhelma". Integr Comp Biol. 42: 1285.
{{cite journal}}
: Unknown parameter|coauthors=
ignored (|author=
suggested) (help) - Northcutt, RG (1981). "Evolution of the telencephalon in nonmammals". Ann Rev Neurosci. 4: 301–50. doi:10.1146/annurev.ne.04.030181.001505. PMID 7013637.
- Northcutt, RG (2008). "Forebrain evolution in bony fishes". Brain Res Bull. 75: 191–205. doi:10.1016/j.brainresbull.2007.10.058. PMID 18331871.
- Parent, A (1995). Carpenter's Human Neuroanatomy. Williams & Wilkins. ISBN 9780683067521.
{{cite book}}
: Unknown parameter|coauthors=
ignored (|author=
suggested) (help) - Paus, T (2001). "Maturation of white matter in the human brain: a review of magnetic resonance studies". Brain Res Bull. 54: 255–66. doi:10.1016/S0361-9230(00)00434-2. PMID 11287130.
{{cite journal}}
: Unknown parameter|coauthors=
ignored (|author=
suggested) (help) - Attention: This template ({{cite pmid}}) is deprecated. To cite the publication identified by PMID 17544173, please use {{cite journal}} with
|pmid=17544173
instead. - Piccolino, M (2002). "Fifty years of the Hodgkin-Huxley era" (PDF). Trends Neurosci. 25: 552–3. doi:10.1016/S0166-2236(02)02276-2. PMID 12392928. Retrieved 2008-10-24.
- Puelles, L (2001). "Thoughts on the development, structure and evolution of the mammalian and avian telencephalic pallium". Philos Trans R Soc Lond B Biol Sci. 356: 1583–98. doi:10.1098/rstb.2001.0973. PMID 11604125.
- Purves, D (1985). Principles of Neural Development. Sinauer Associates. ISBN 9780878937448.
{{cite book}}
: Unknown parameter|coauthors=
ignored (|author=
suggested) (help) - Raichle, M (2002). "Appraising the brain's energy budget". PNAS. 99: 10237–9. doi:10.1073/pnas.172399499. PMID 12149485.
{{cite journal}}
: Unknown parameter|coauthors=
ignored (|author=
suggested) (help) - Reiner, A (2005). "Organization and evolution of the avian forebrain". Anat Rec a Discov Mol Cell Evol Biol. 287: 1080–102. PMID 16206213. Retrieved 2008-10-12.
{{cite journal}}
: Unknown parameter|coauthors=
ignored (|author=
suggested) (help) - Ridley, M (2003). Nature via Nurture: Genes, Experience, and What Makes Us Human. Forth Estate. ISBN 9780060006785.
- Safi, K (2005). "Bigger is not always better: when brains get smaller". Biol Lett. 1: 283–6. doi:10.1098/rsbl.2005.0333. PMID 17148188.
{{cite journal}}
: Unknown parameter|coauthors=
ignored (|author=
suggested) (help); Unknown parameter|pmcid=
ignored (|pmc=
suggested) (help) - Saitoh, K (2007). "Tectal control of locomotion, steering, and eye movements in lamprey". J Neurophysiol. 97: 3093–108. doi:10.1152/jn.00639.2006. PMID 17303814.
{{cite journal}}
: Unknown parameter|coauthors=
ignored (|author=
suggested) (help) - Salas, C (2003). "Evolution of forebrain and spatial cognition in vertebrates: conservation across diversity". Brain Behav Evol. 62: 72–82. doi:10.1159/000072438.
{{cite journal}}
: Unknown parameter|coauthors=
ignored (|author=
suggested) (help) - Savage, MV (2004). "The predominance of quarter-power scaling in biology". Functional Ecol. 18: 257–82. doi:10.1111/j.0269-8463.2004.00856.x.
{{cite journal}}
: Unknown parameter|coauthors=
ignored (|author=
suggested) (help) - Schmidt-Rhaesa, A (2007). The Evolution of Organ Systems. Oxford University Press. ISBN 9780198566694.
- Searle, J (1999). Mind, Language and Society. Basic Books. ISBN 9780465045211.
- Shepherd GM (1994). Neurobiology. Oxford University Press. ISBN 9780195088434.
- Shin, HS (1985). "An unusual coding sequence from a Drosophila clock gene is conserved in vertebrates". Nature. 317: 445–8. doi:10.1038/317445a0. PMID 2413365.
{{cite journal}}
: Unknown parameter|coauthors=
ignored (|author=
suggested) (help) - Shu DG, Morris SC, Han J, Zhang Z-F; et al. (2003). "Head and backbone of the Early Cambrian vertebrate Haikouichthys". Nature. 421: 526–529. doi:10.1038/nature01264. PMID 12556891.
{{cite journal}}
: Explicit use of et al. in:|author=
(help)CS1 maint: multiple names: authors list (link) - Storrow, HA (1969). Outline of Clinical Psychiatry. Appleton-Century-Crofts. ISBN 9780390850751.
- Striedter, GF (2005). Principles of Brain Evolution. Sinauer Associates. ISBN 9780878938209.
- Swaab, DF (2003). The Human Hypothalamus. Elsevier. ISBN 9780444513571.
{{cite book}}
: Unknown parameter|coauthors=
ignored (|author=
suggested) (help) - Tonegawa, S (2003). "Genetic neuroscience of mammalian learning and memory". Philos Trans R Soc Lond B Biol Sci. 358: 787–95. doi:10.1098/rstb.2002.1243. PMID 12740125.
{{cite journal}}
: Unknown parameter|coauthors=
ignored (|author=
suggested) (help) - van Hemmen, JL (2005). 23 Problems in Systems Neuroscience. Oxford University Press. ISBN 9780195148220.
{{cite book}}
: Unknown parameter|coauthors=
ignored (|author=
suggested) (help) - van Praag, H (2000). "Neural consequences of environmental enrichment". Nat Rev Neurosci. 1: 191–8. PMID 11257907.
{{cite journal}}
: Unknown parameter|coauthors=
ignored (|author=
suggested) (help) - von Neumann, J (2000). The computer and the brain. Yale Univ. Press. ISBN 0-300-08473-0.
{{cite book}}
: Unknown parameter|coauthors=
ignored (|author=
suggested) (help) - Wiesel, T (1982). "Postnatal development of the visual cortex and the influence of environment" (PDF). Nature. 299: 583–91. doi:10.1038/299583a0. PMID 6811951.
- White, JG (1986). "The Structure of the Nervous System of the Nematode Caenorhabditis elegans". Phil Trans Roy Soc London (Biol). 314: 1–340. doi:10.1098/rstb.1986.0056.
{{cite journal}}
: Unknown parameter|coauthors=
ignored (|author=
suggested) (help) - Willmer P (1990). Invertebrate Relationships: Patterns in Animal Evolution. Cambridge University Press. ISBN 9780521337120.
- Wong, R (1999). "Retinal waves and visual system development". Ann Rev Neurosci. 22: 29–47. doi:10.1146/annurev.neuro.22.1.29. PMID 10202531.
- Wyllie, E (2005). The Treatment of Epilepsy: Principles and Practice. Lippincott Williams & Wilkins. ISBN 9780781749954.
{{cite book}}
: Unknown parameter|coauthors=
ignored (|author=
suggested) (help) - "Flybrain: An online atlas and database of the drosophila nervous system".
- "WormBook: The online review of c. elegans biology".
Further reading
- Bear, Mark F. (2006). Neuroscience. Philadelphia, Pennsylvania: Lippincott Williams & Wilkins. ISBN 9780781760034. OCLC 62509134.
{{cite book}}
: Unknown parameter|coauthors=
ignored (|author=
suggested) (help) - Blackmore, Susan M. (2006). Conversations on Consciousness. Oxford; New York: Oxford University Press. ISBN 9780195179583. OCLC 62555307.
- Buzsaki, Gyorgy (2006). Rhythms of the Brain. Oxford; New York: Oxford University Press. ISBN 9780195301069. OCLC 63279497.
- Calvin, William H. (2001). The River That Flows Uphill: A Journey from the Big Bang to the Big Brain. Lincoln, Nebraska: Iuniverse.com. ISBN 9780595167005. OCLC 48962546.
- Della Sala, Sergio (1999). Mind myths: Exploring popular assumptions about the mind and brain. Chichester England; New York: J. Wiley & Sons. ISBN 0471983039. OCLC 39700332.
- Restak, Richard (2001). The Secret Life of the Brain. Washington, DC: Joseph Henry Press. ISBN 9780309074353. OCLC 47863192.
- Shepherd, Gordon M. (2004). The Synaptic Organization of the Brain (Fifth ed.). Oxford; New York: Oxford University Press. ISBN 9780195159561. OCLC 51769076.
Written for children 8 and older:
- Simon, Seymour (2000). The Brain. New York: Morrow Junior. ISBN 9780688170608. OCLC 35686089.
External links
- The Society for Neuroscience
- International Brain Research Organization
- The HOPES Brain Tutorial at hopes.stanford.edu
- Comparative Mammalian Brain Collection
- Brain Research News from ScienceDaily
- BrainInfo for Neuroanatomy
- Neuroscience for kids
- BrainMaps.org, interactive high-resolution digital brain atlas based on scanned images of serial sections of both primate and non-primate brains
- The Brain from Top to Bottom
- The Department of Neuroscience at Wikiversity
- The Secret Life of the Brain : History of the Brain from PBS
- University of Washington 3D animations of brain regions - click through from "Click for copyright"